Smart Polymer Brushes
This is a collaborative project with external page Dr. Celestino Padeste’s group at the Paul Scherrer Institute.
Smart surfaces are of great interest due to their stimulus-induced switching of properties on demand. Switching originates from responsiveness to a certain trigger such as temperature, pH, redox potential, mechanical stress, ionic strength, solvents, an external electric field or light.
Micro- and Nanostructured Polymer Brushes[1, 2, 3, 4]
Patterns of radicals serving as initiators are created by exposure to extreme ultraviolet (EUV)-light using the X‑ray interference lithography beamline at the Swiss Light Source. Depending on the diffraction mask used, various periodic brush structures with resolution in the micrometer to nanometer scale can be produced.
The EUV lithography setup for the creation of nano‑patterned polymer brushes is shown in Figure 1. The photon energy of the undulator light is 92.5 eV (λ = 13.5 nm), which is well suited for cracking chemical bonds and therefore creating radicals on polymer surfaces. Patterns on the surface of polymer foils are generated by interference of beams diffracted at grating structures. Using these radicals as initiators, micro- and nanostructured polymer brushes are covalently grafted from substrate polymer surfaces. Exposures through a typical mask with diffraction gratings lead to line arrays with a period from 1 µm down to 100 nm for two interfering beams and to dot arrays with a period from 1.4 µm down to 140 nm, respectively. The size of the patterned fields for small-period patterns is in the range of 200 µm2.
Atomic force microscopy (AFM) images of areas covered with brush nanostructures grafted using free radical polymerization (FRP) are shown in Figure 2. Patterns were defined by two, four or six interfering EUV‑beams using the established lithography setup. Two interfering EUV-beams led to nano-patterned line structures, four interfering EUV-beams led to dot structures, and six interfering EUV-beam led to hexagonal structures. Homogeneous copolymer hexagonal nano‑patterned structures with a strong contrast could be grafted, as indicated also in the cross-section profile. In this case cavities of 100-200 nm in diameter were formed by dots six hexagonally arranged of 650 nm in width.
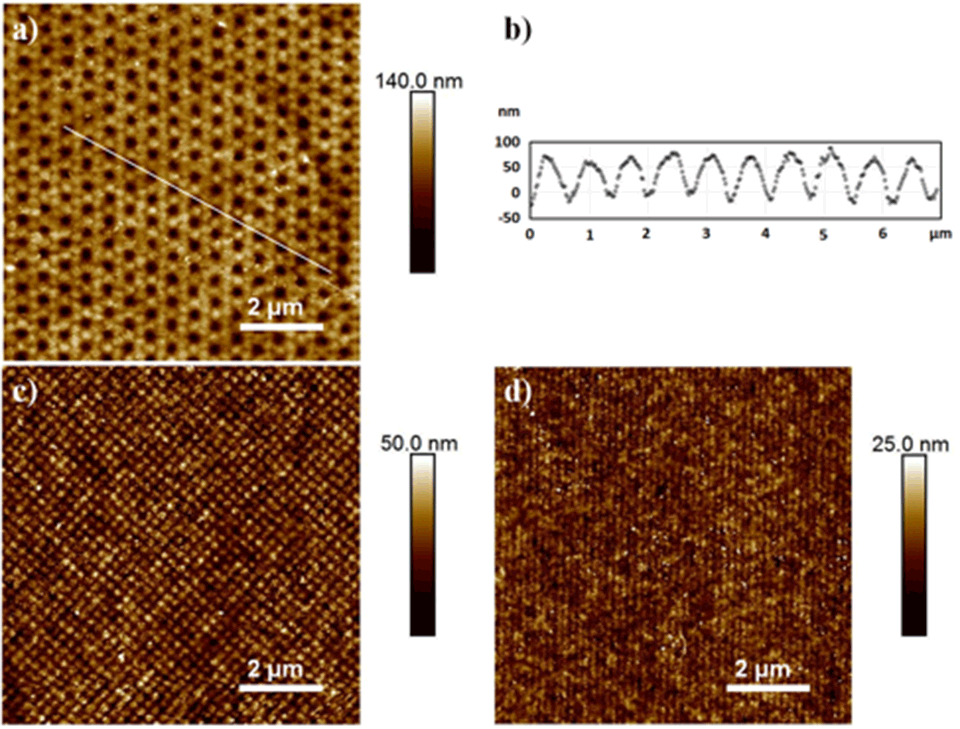
Light-Responsive Polymer Brushes[3]
New strategies to form photochromic spiropyran (SP)-containing polymer brushes on polymer surfaces via post‑polymerization modification are demonstrated. Brushes are grafted from the surface of polymer foils by free‑radical polymerization (FRP). The photochromism of spiropyrans has been studied intensively since the 1950s, in which a heterocycle and a chromene moiety are orthogonally linked through a spiro‑carbon atom. It is based on the reversible transformation of the ring‑closed colorless spiropyran (SP) and two planar canonical open forms-zwitterionic and uncharged-of the deeply colored merocyanine (MC) (Figure 3). The heterolytic cleavage of the sp3 carbon‑oxygen bond in the pyran ring is caused by UV light and can be reversed back to the thermodynamically stable form upon heating or irradiation with visible light.
Grafted surfaces were generated via plasma activation showing a reversible switch in static water contact angle of up to 15° for PGMA‑SP and up to 30° for PMA-SP brushes, upon alternating visible and UV-light irradiation (Figure 4). The switch in CA for SP-containing brushes is strongly dependent on the chemical environment provided by the brush. The substrate on the other hand seems not to play a role in terms of the surface free energy for each modification step, as the values are equal within the error range for ETFE and PTFE. This shows that the post-polymerization modification approaches work universally on different fluorocarbon polymer substrates and could also, in principle, be transferred to other polymers, such as polyolefins.
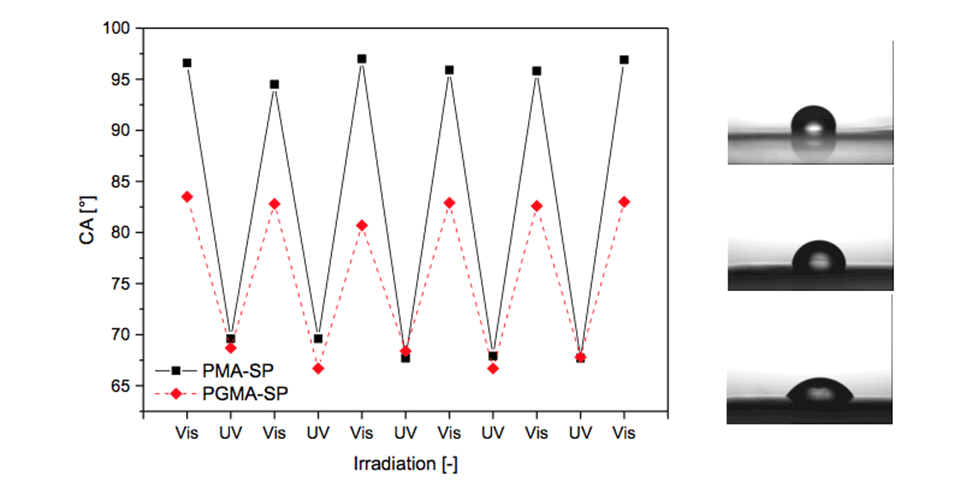
The fluorescence-emission kinetics of patterns of SP‑containing brushes were analyzed in the dry state and in solvents of different polarities using fluorescence microscopy (Figure 5). Again, upon an initial excitation by UV‑light (λEx = 360 nm, 30 s) the open MC is formed, which can then be excited via subsequent continuous irradiation using a second excitation wavelength (λEx = 555 nm). This excitation leads to an emission of red fluorescence (λEm = 630 nm) on the generated arrays of micro- and nano-patterned polymer brushes. As a consequence of the much higher brush thickness in the dry state - and therefore the higher number of binding sites - the areas of high radical density show stronger fluorescence emission. However, the periodic interference patterns also show a clear - albeit much weaker - emission.
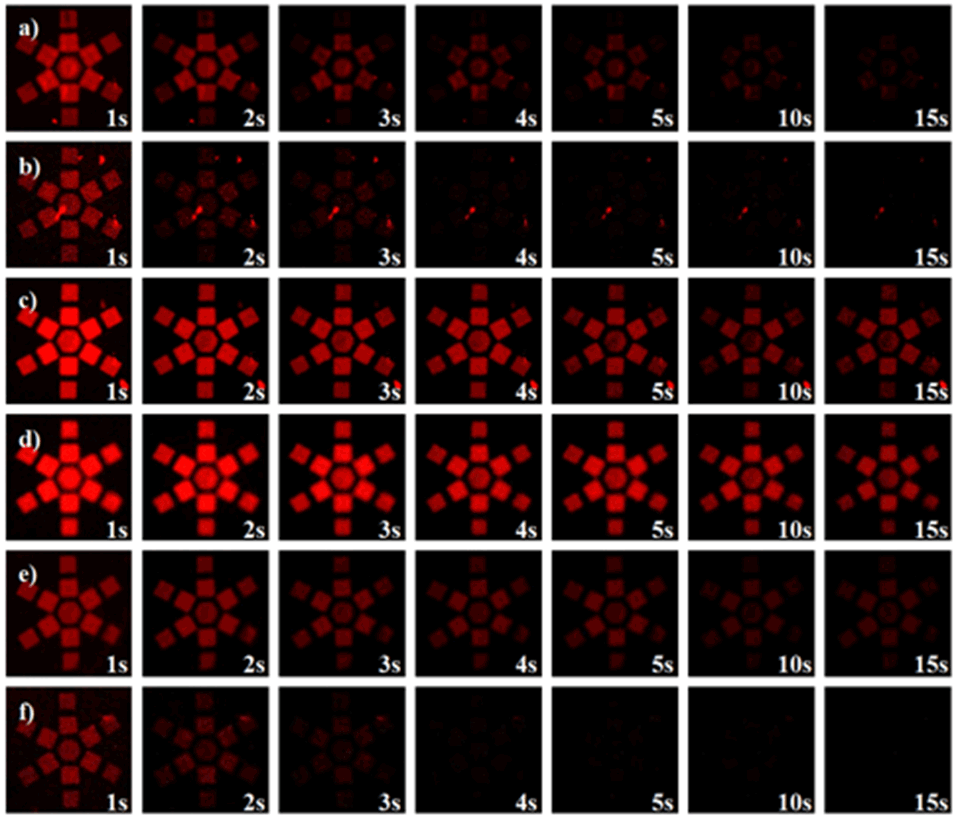
‘Clickable’ Copolymer-Brushes[4]
Patterned functional polymeric surfaces with reactive groups that can be modified under mild conditions without employing any metal catalyst open a broad field of applications and are of particular interest for the bioconjugation of polymeric materials. Such patterns need to be fabricated with properties and characteristics that enable them to interact with their environment in a desired manner. Polymer brushes are ideal candidates for such applications, since polymers can be readily synthesized to be multifunctional by the incorporation of several monomers with different properties and functions.
A new approach to grafting thiol-reactive nano-patterned copolymer-brush structures on polymeric substrates by means of EUV-IL was demonstrated. The copolymer brushes were designed to contain maleimide functional groups as thiol-reactive centers. The number of reactive centers on the grafted brush structures can be tailored by varying the monomer ratios in the feed. Thiol-containing moieties were conjugated using the nucleophilic Michael-addition reaction, which proceeds at room temperature without the need for any metal-based catalyst. Using this approach, a variety of functionalities was introduced to yield polyelectrolytes, as well as fluorescent and light-responsive polymer-brush structures (Figure 7).
Smart Membranes[5]
Smart membranes that are sensitive to their environment have received tremendous attention in recent years as they enable the rapid, remote-controlled switching of their interfacial properties. They are designed materials with properties such as swelling behavior, permeability or interactions with ions and biomolecules that can be changed reversibly in a controlled way by means of external stimulation. Switching originates from responsiveness to a certain trigger such as changes in pH, temperature, concentration of metal ions or exposure to light.
A new method for the fabrication of responsive polymeric membranes has been demonstrated that uses argon-plasma activation and free‑radical polymerization to graft pH-responsive PMAA polymer brushes from microporous polypropylene (Figure 8). It was clearly evident from ATR-IR spectra that PMAA polymer brushes had been grafted and functionalized via a two-step post-polymerization modification strategy to generate photochromic spiropyran-containing PMA-SP polymer brushes. Both PMAA and PMA-SP modified membranes were successfully shown to be smart materials that could switch in wettability and permeability in response to either pH or light as external stimulus. Switching was demonstrated by means of static water-contact angle and water-flux measurements in dependence on their grafting level. In addition, photochromic PMA-SP brushes show a reversible switch in color upon alternating visible and UV-light irradiation. The flux properties of pH-switched PMAA-modified membranes were dominated by the swelling of the brushes and little influenced by the hydrophilicity of the surface (Figure 9, top). In contrast, hydrophilicity changes dominated the photon-induced switching of SP-modified membranes (Figure 9, bottom). The advantage of the presented approach lies in its simplicity and versatility. The application of responsive systems to influence the hydrophilicity or pore size via swelling of the material is of great interest for the controlled separation or transport of dissolved species.
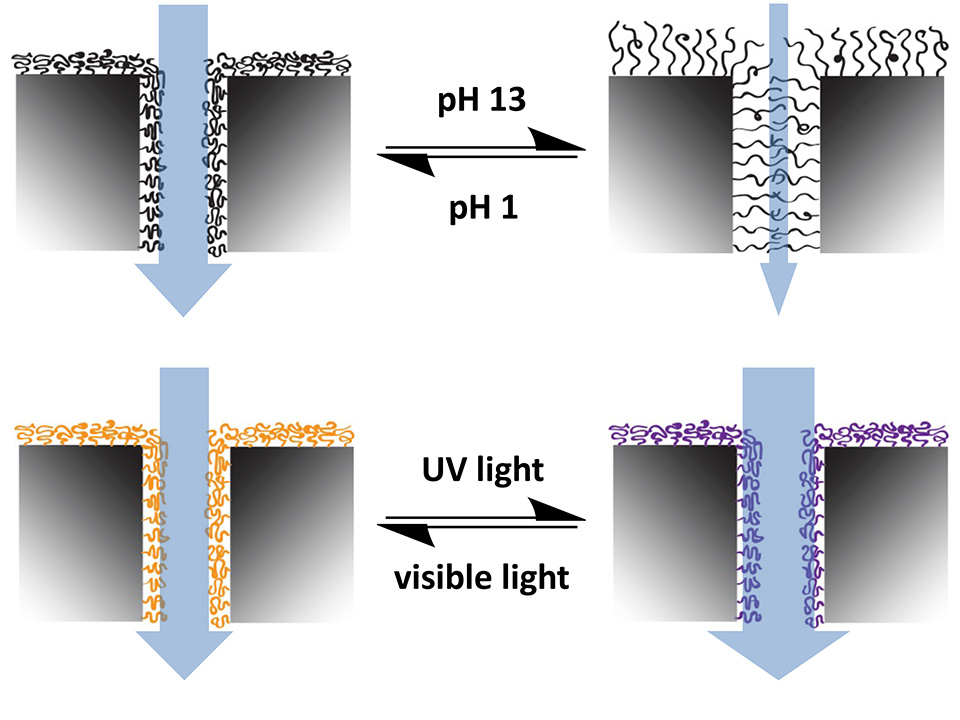
Reversible Light-Switching of Enzymatic Activity[6]
UV- and visible light-induced switching of enzymatic activity has been demonstrated using surface-grafted polymer brushes functionalized with microperoxidase MP-11 and spiropyran moieties. Integration into an optofluidic device allowed reversible switching of the enzymatic activity under flow (Figure 10).
A polymeric brush biointerface that enables light-switching of an enzymatic reaction was realized by means of orthogonal functionalization of polymeric substrates with amine- and thiol-reactive copolymer brushes. Facile and effective amine-epoxide and thiol-ene conjugation were employed as a toolbox for sequential and chemospecific binding of enzymatically active MP-11 and light-responsive SP to grafted brushes (Figure 11). The combination of both functionalities in a single brush allowed us to reversibly switch the enzymatic activity using light as an external stimulus.
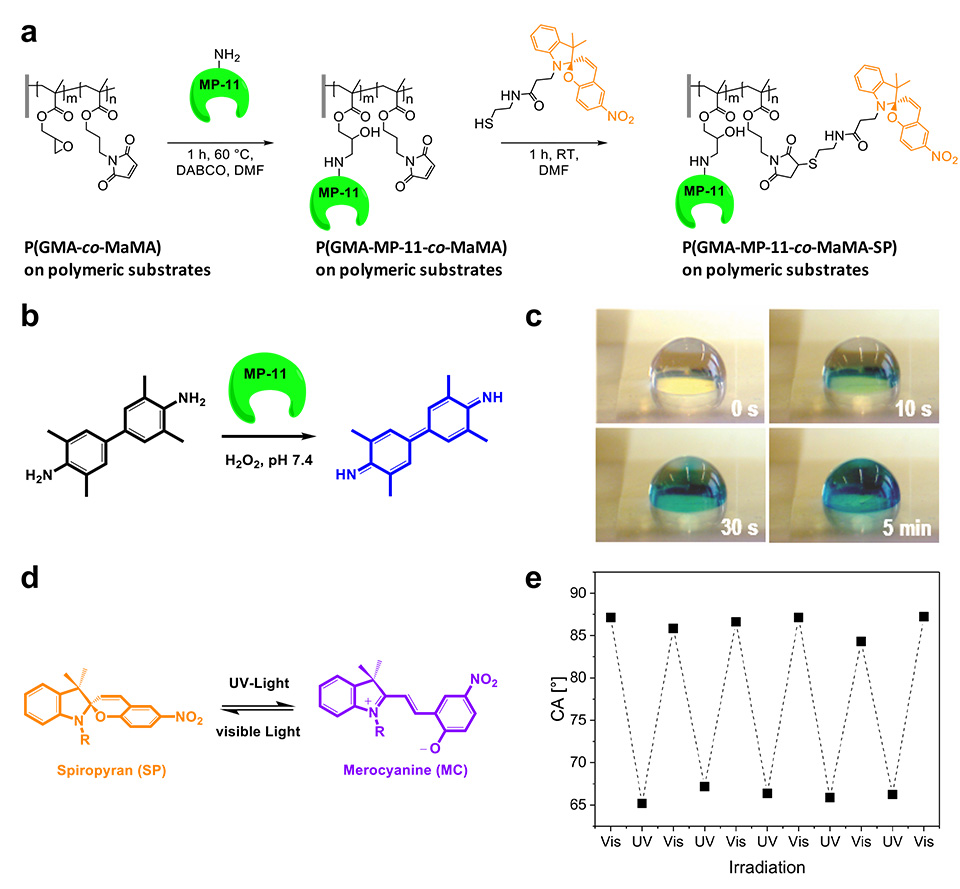
In addition, integration of multifunctional polymer brushes grafted from ETFE into an optofluidic device allowed reversible switching of the enzymatic turn-over of TMB under flow (Figure 12). This enabled the dynamic enzymatic activity of the bioconjugated microchannel to be controlled in an all-polymeric photonic lab-on-a-chip system. The results represent a major milestone in responsive bioconjugated polymeric surfaces, as they combine chemospecific highly efficient PPM in an orthogonal fashion to implement multifunctionality on a single brush. This new type of synthetic platform demonstrates the proof of concept with the chosen enzyme MP-11, being an exemplary biocatalyst. The well-controlled brush structures open up a broad variety of applications for responsive bioconjugated polymer brushes in, for example, all-polymeric smart diagnostic systems in which metabolic events can be in investigated in well-defined areas of interest.
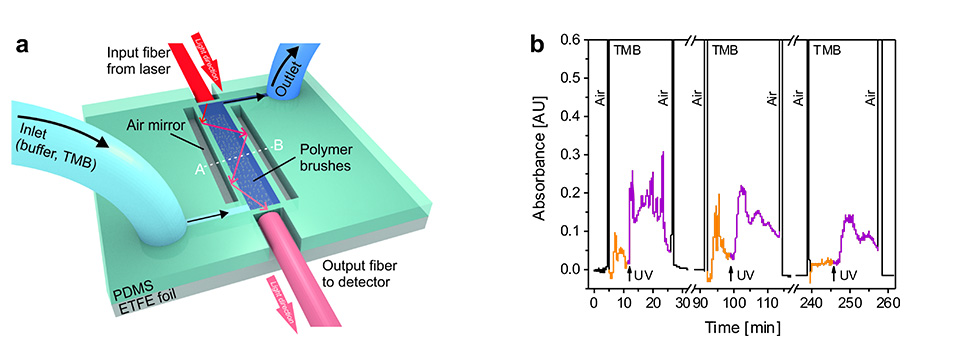
References
[1] S. Neuhaus, C. Padeste, H.H. Solak, N.D. Spencer, Polymer 2010, 51, 4037-4043.
[2] C. Padeste, S. Neuhaus, Polymer Micro- and Nanografting, Elsevier 2015.
[3] M. Dübner, N.D. Spencer, C. Padeste, Langmuir 2014, 30, 14971−14981.
[4] M. Dübner, T.N. Gevrek, A. Sanyal, N.D. Spencer, C. Padeste, ACS Appl. Mater. Interfaces 2015, 7, 11337−11345.
[5] M. Dübner, M.-E. Naoum, N.D. Spencer, C. Padeste, ACS Omega 2017, 2, 455−461.
[6] M. Dübner, V.J. Cadarso, T.N. Gevrek, A. Sanyal, N.D. Spencer, C. Padeste. ACS Appl. Mater. Interfaces 2017, 9, 9245−9249.